Carbon, carbon, everywhere, and all in the wrong carbon sink. With apologies to Samuel Taylor Coleridge, this sums up the carbon quandary: on the one hand, we have too much carbon dioxide and methane from fossil fuel sources in our atmosphere. On the other hand, we may not have enough carbon from sustainable sources to drive our transition away from fossil fuels.
The global economies are working to decarbonise and switch to sustainable sources for energy and materials, with the chemicals industry key to addressing that challenge. Some sectors will be harder to decarbonise than others, and carbon-rich molecules will be needed both to enable the net zero transition and in the longer term. Yet the pace of change that is needed, in every country and across all sectors, puts tight constraints on the resources available.
These constraints create one of the central science and technology challenges we face: producing 100% renewable liquid energy
The chemicals sector, for example, is said to be responsible for about 5% of global CO2 emissions.1 To reduce that figure it has to decarbonise its raw materials, its chemical making processes, its chemical product making and usage, and also its waste and recycling. Many of these are inherently carbon rich and often energy intensive by way of high-temperature heat. Considered in isolation, low carbon feedstocks and clean energy are the solutions here.
Yet the rub of the net zero and decarbonisation challenge is that it cannot be reduced so simply; it is a problem that brings social, ecological, technical and economic factors together in a complex, interlinked system. That means an individual sector, such as chemicals, must include externalities in environmental areas such as land-use and water resource alongside supply chain issues, such as the critical minerals needed for catalysts, and societal factors such as demand change.
Taking a systems thinking approach to the problem, these constraints create what is, in my opinion, one of the central science and technology challenges that we face: producing 100% renewable liquid energy that closes the loop on combustion.
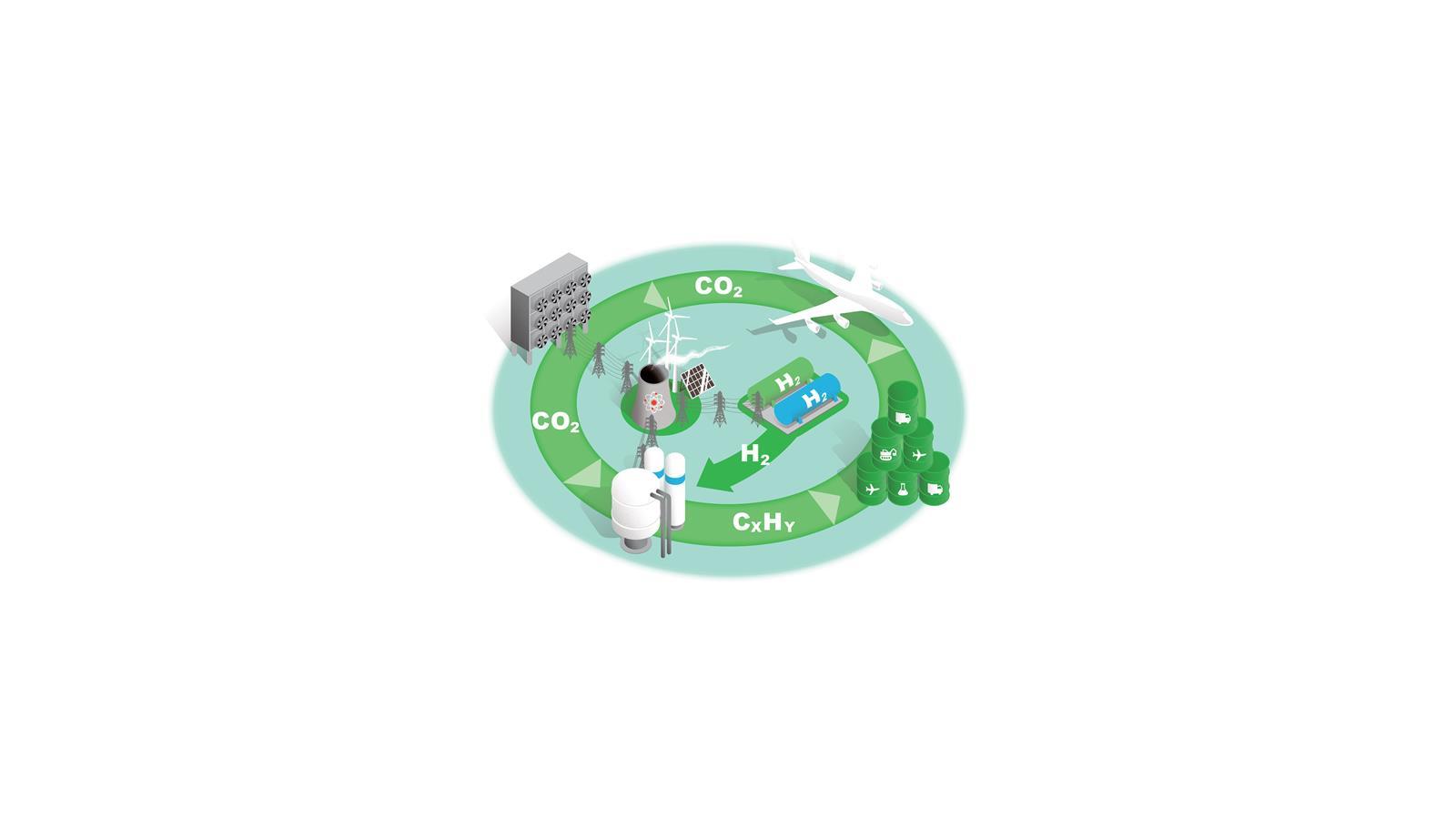
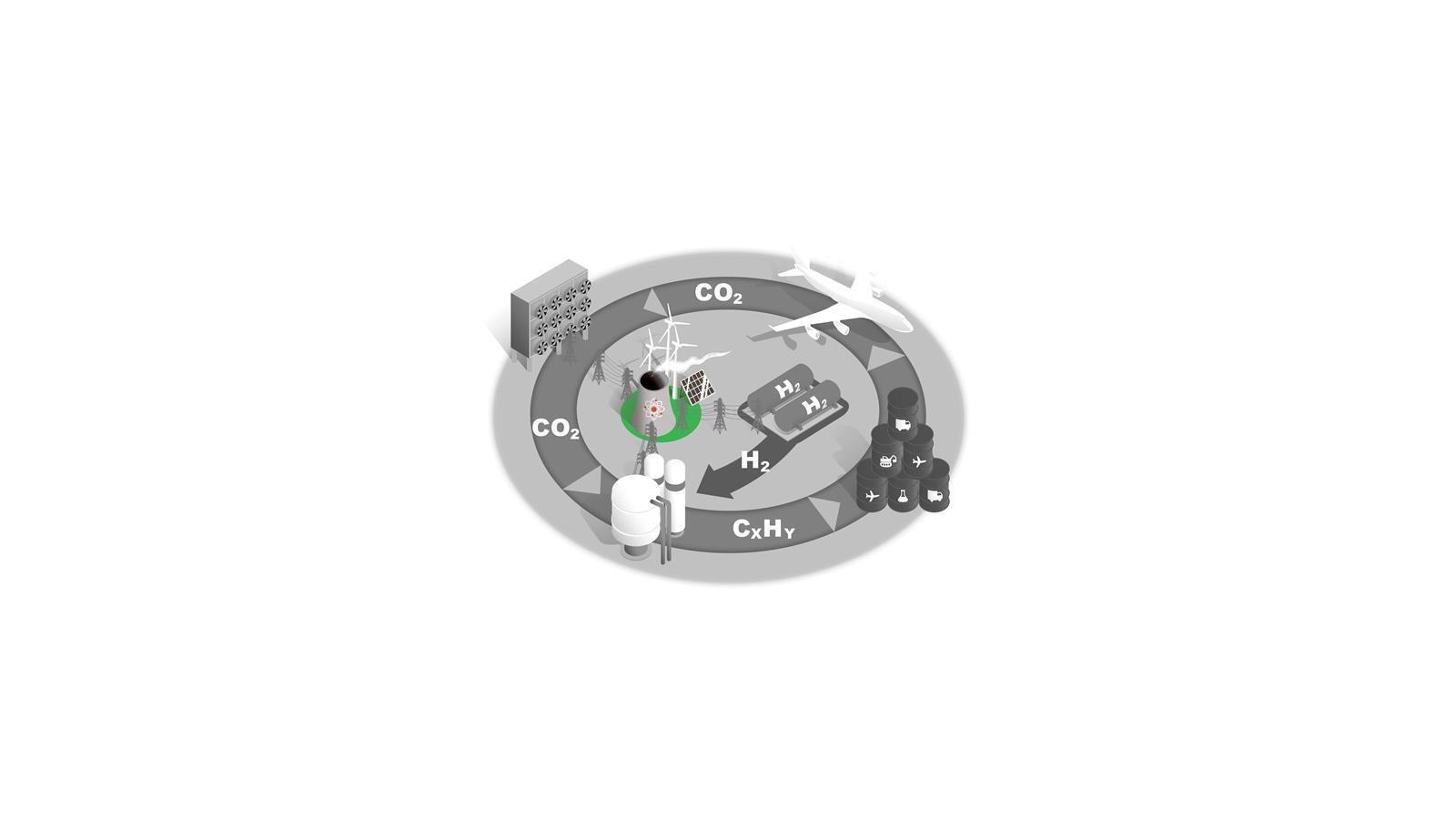
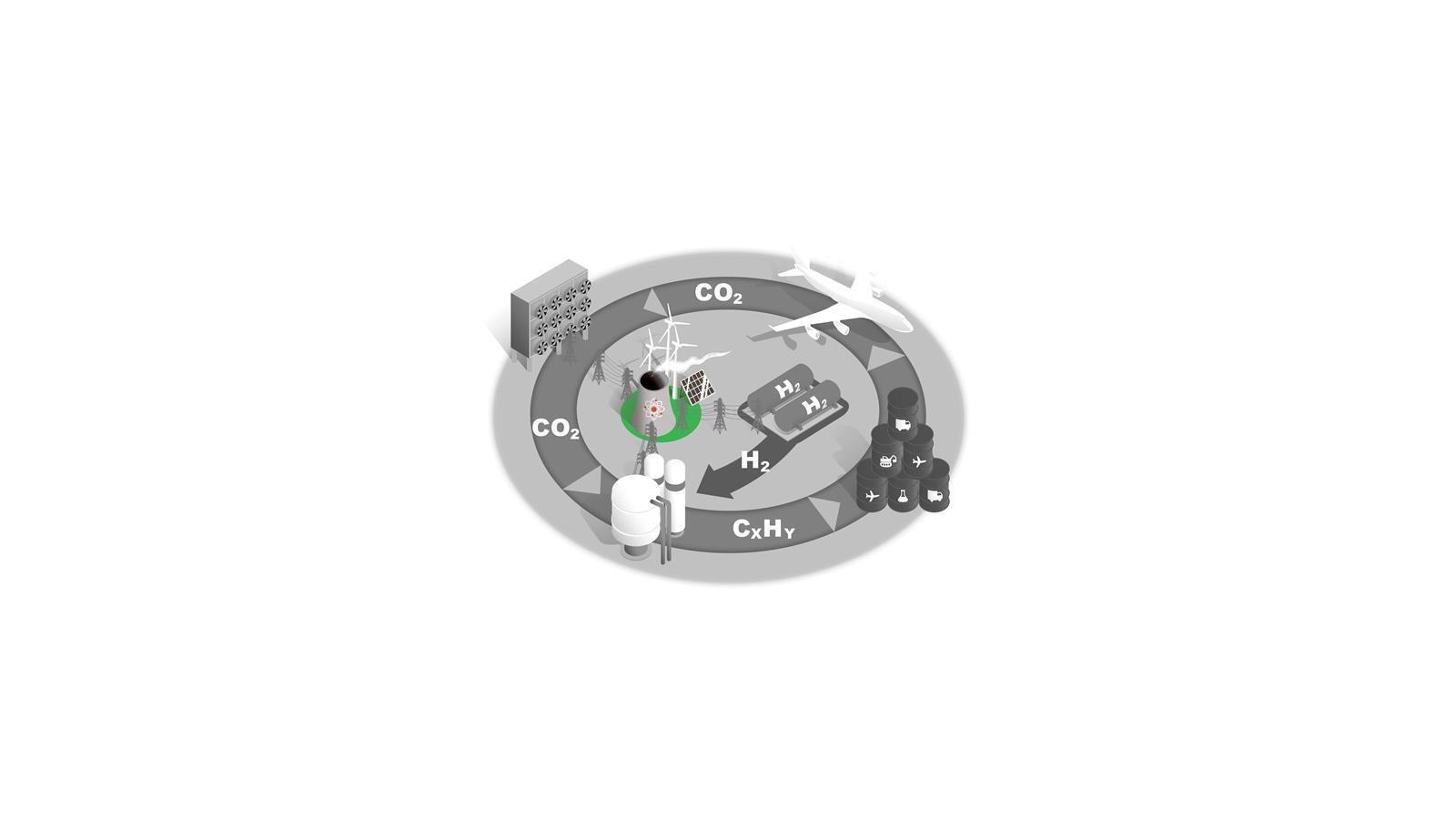
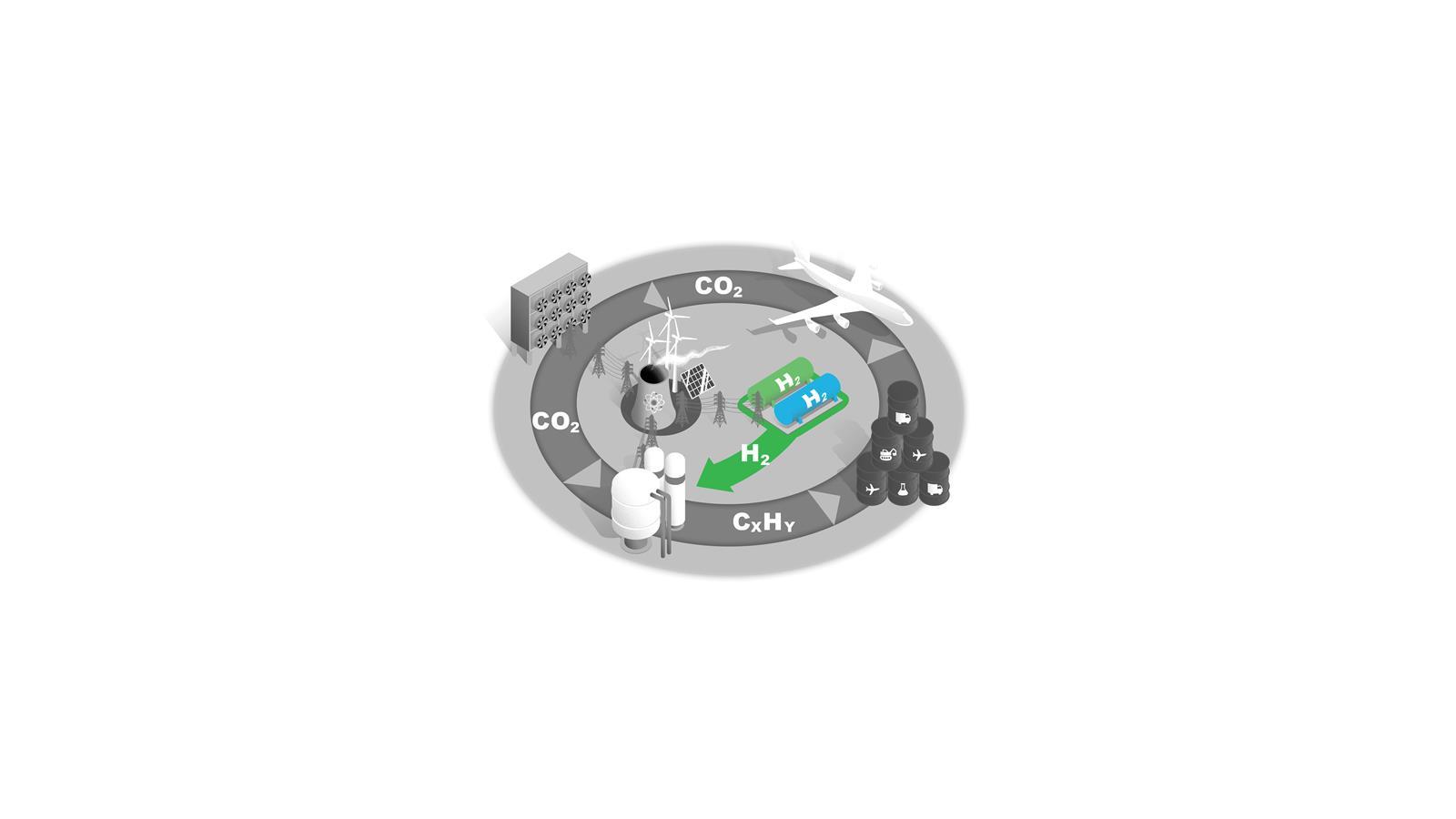
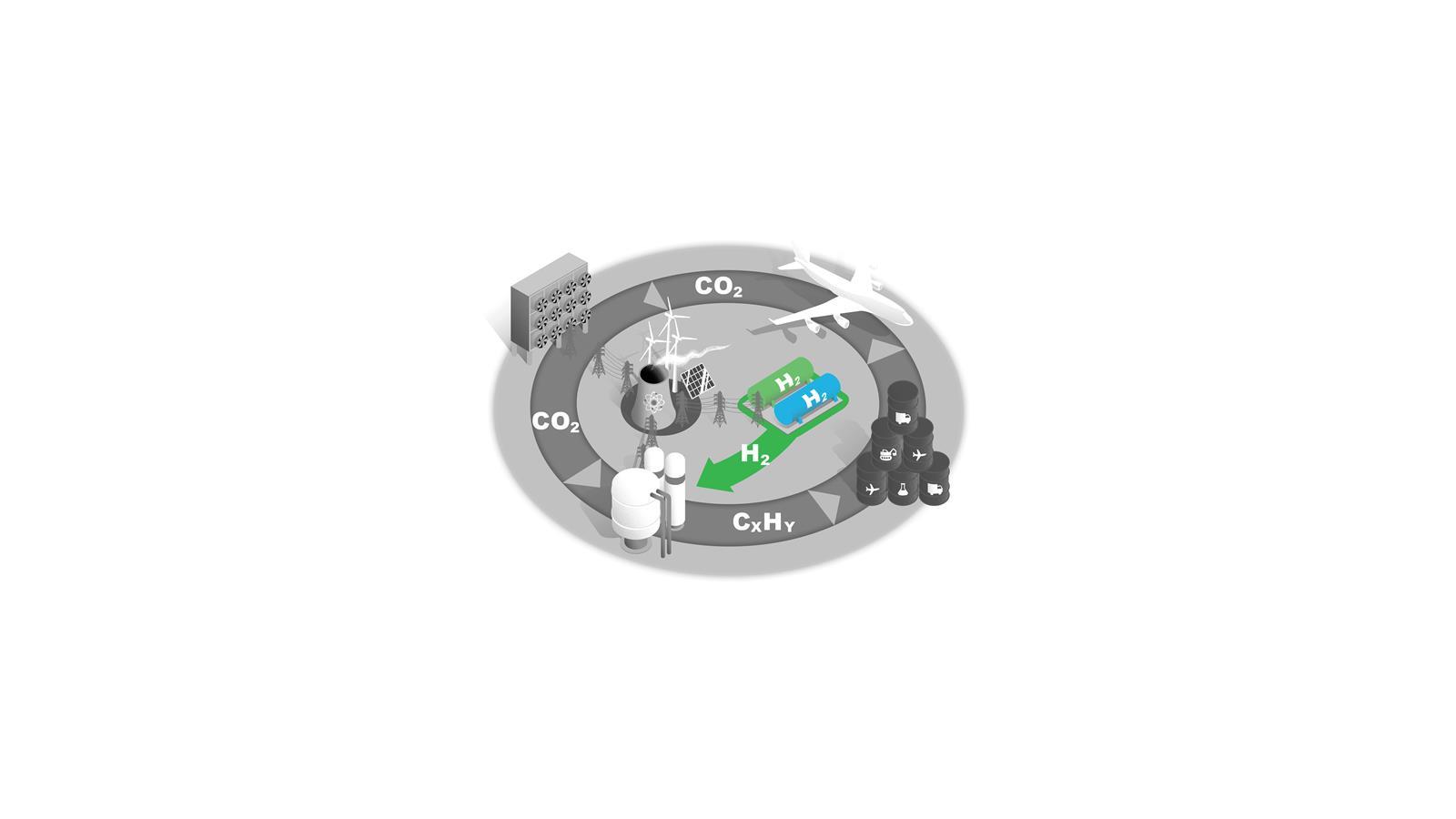
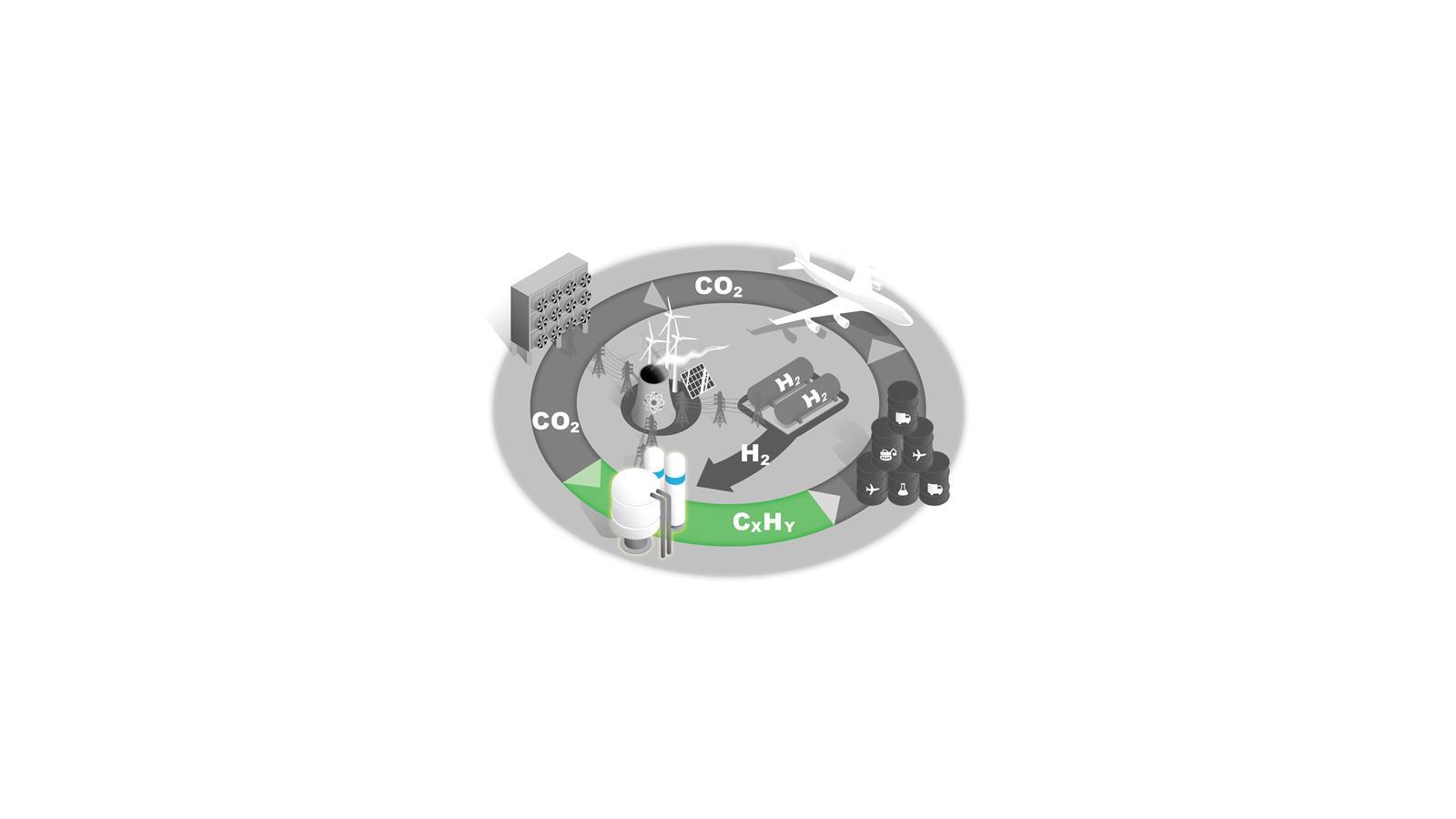
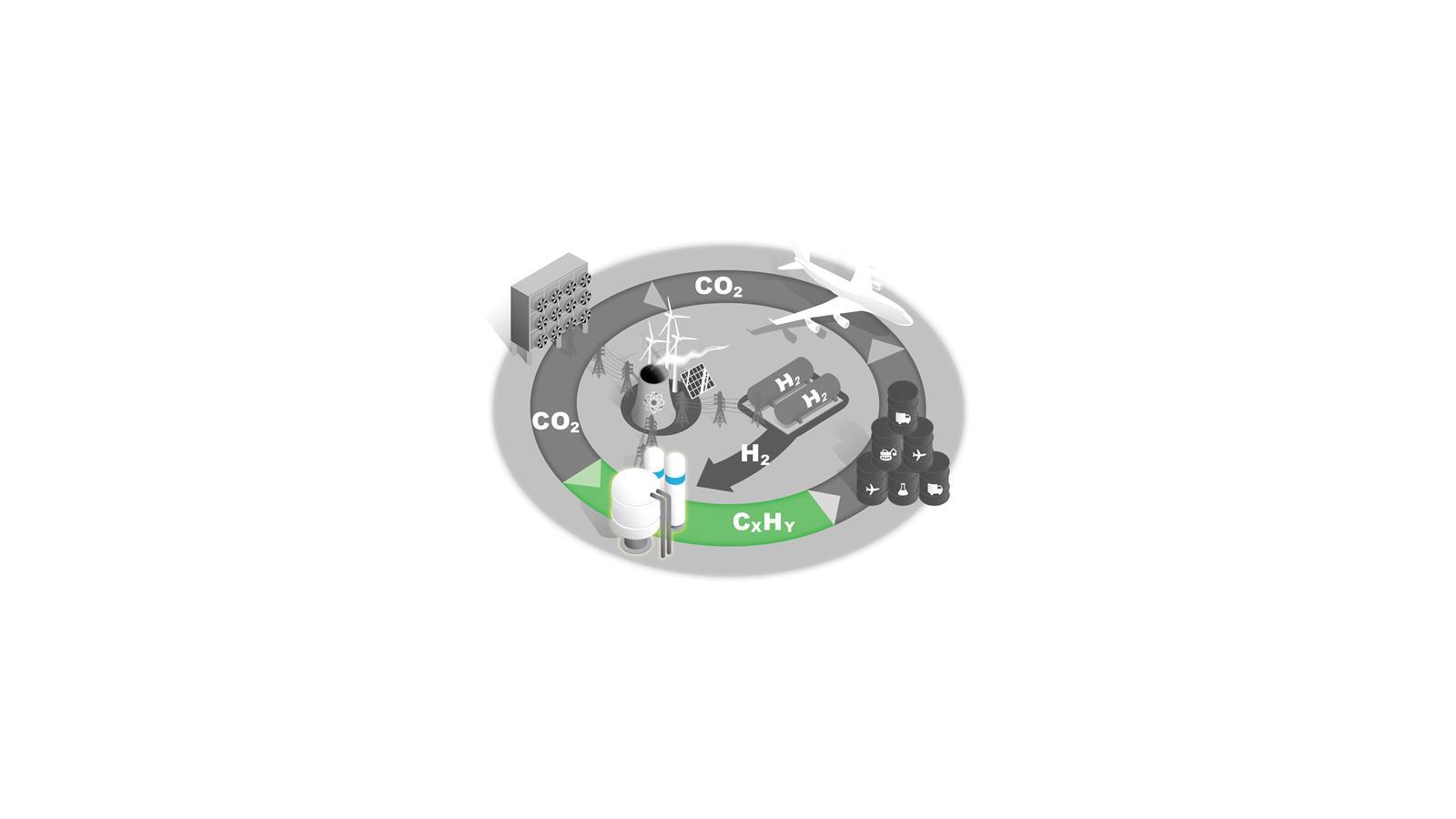
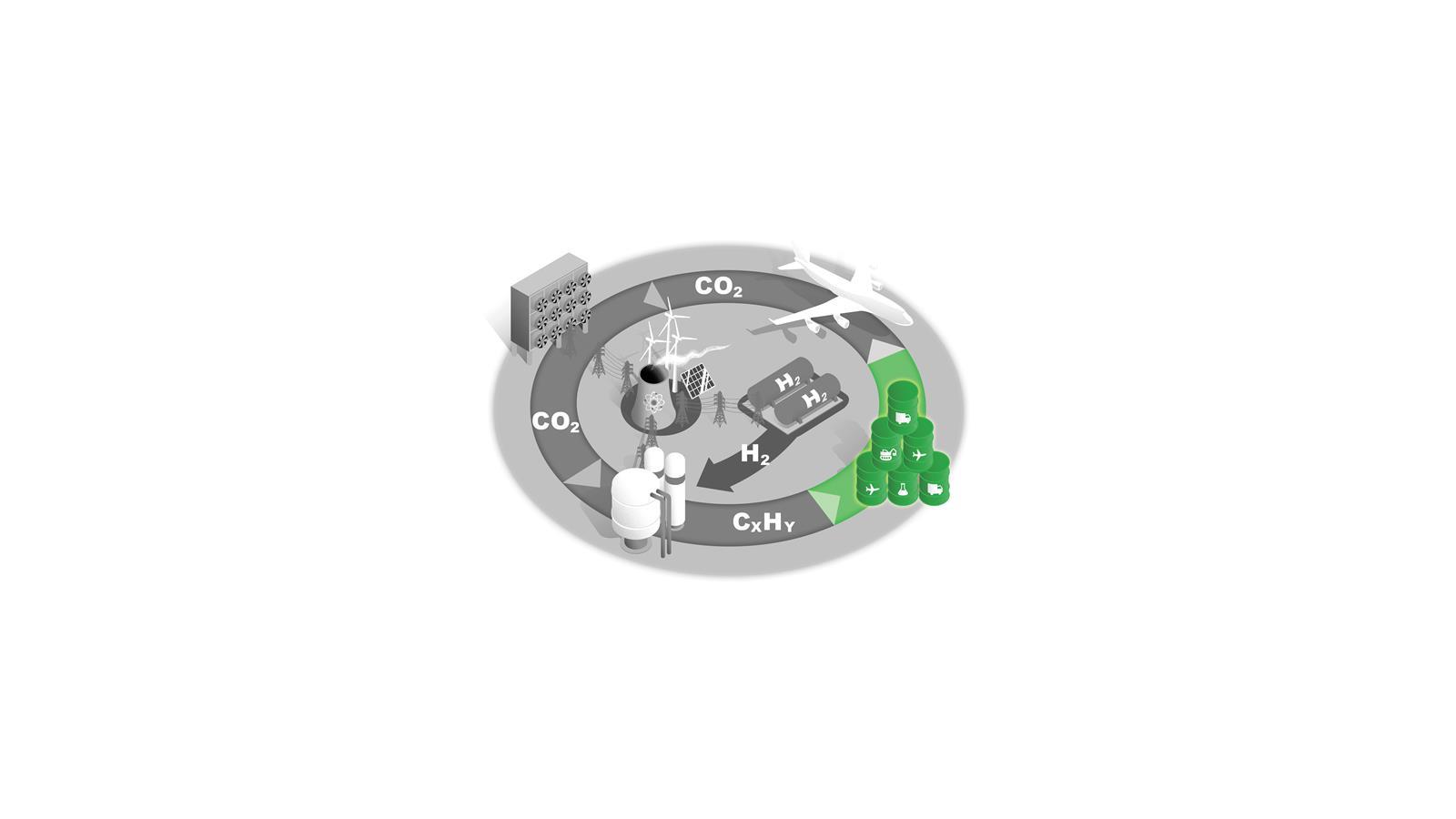
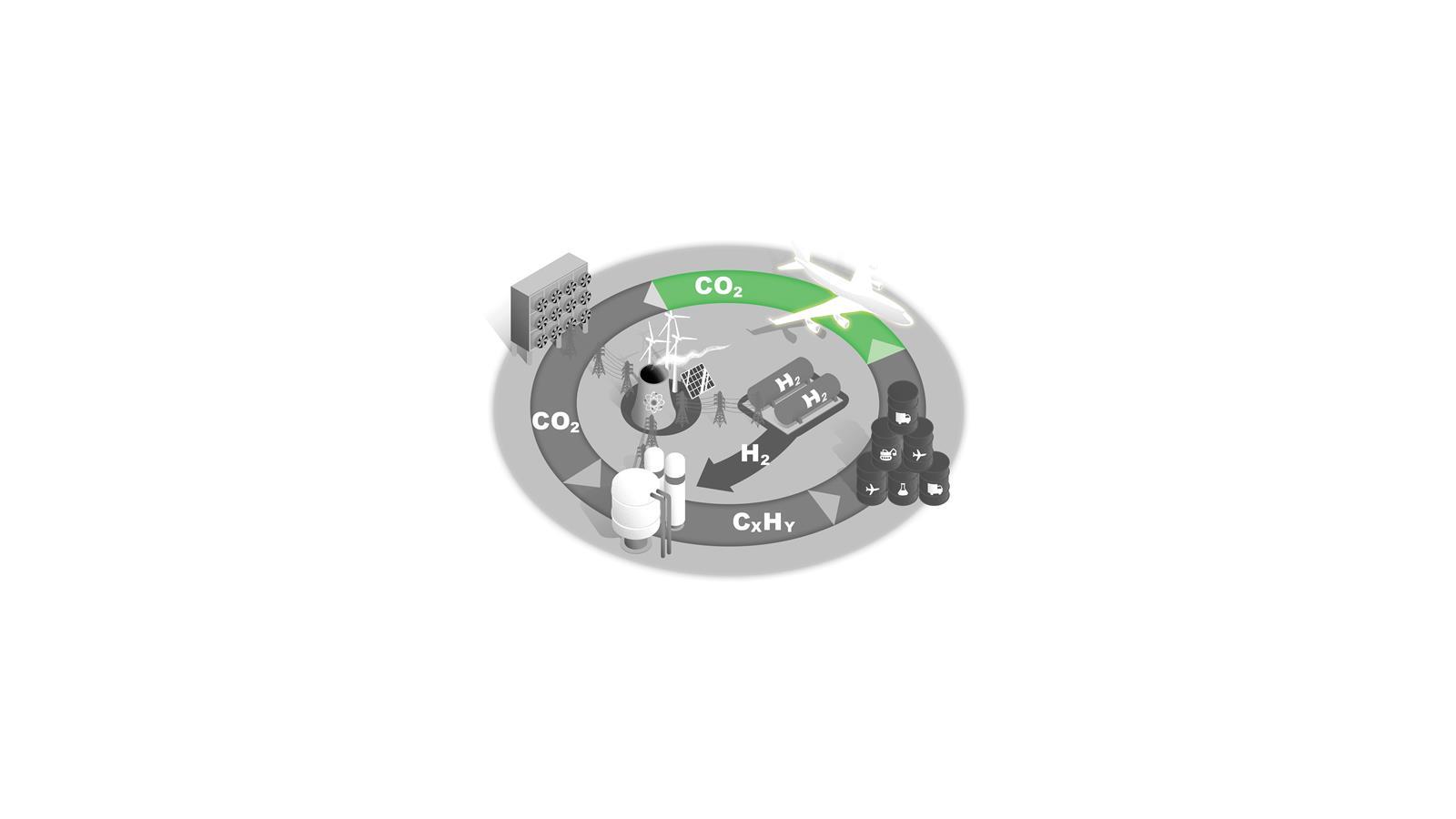
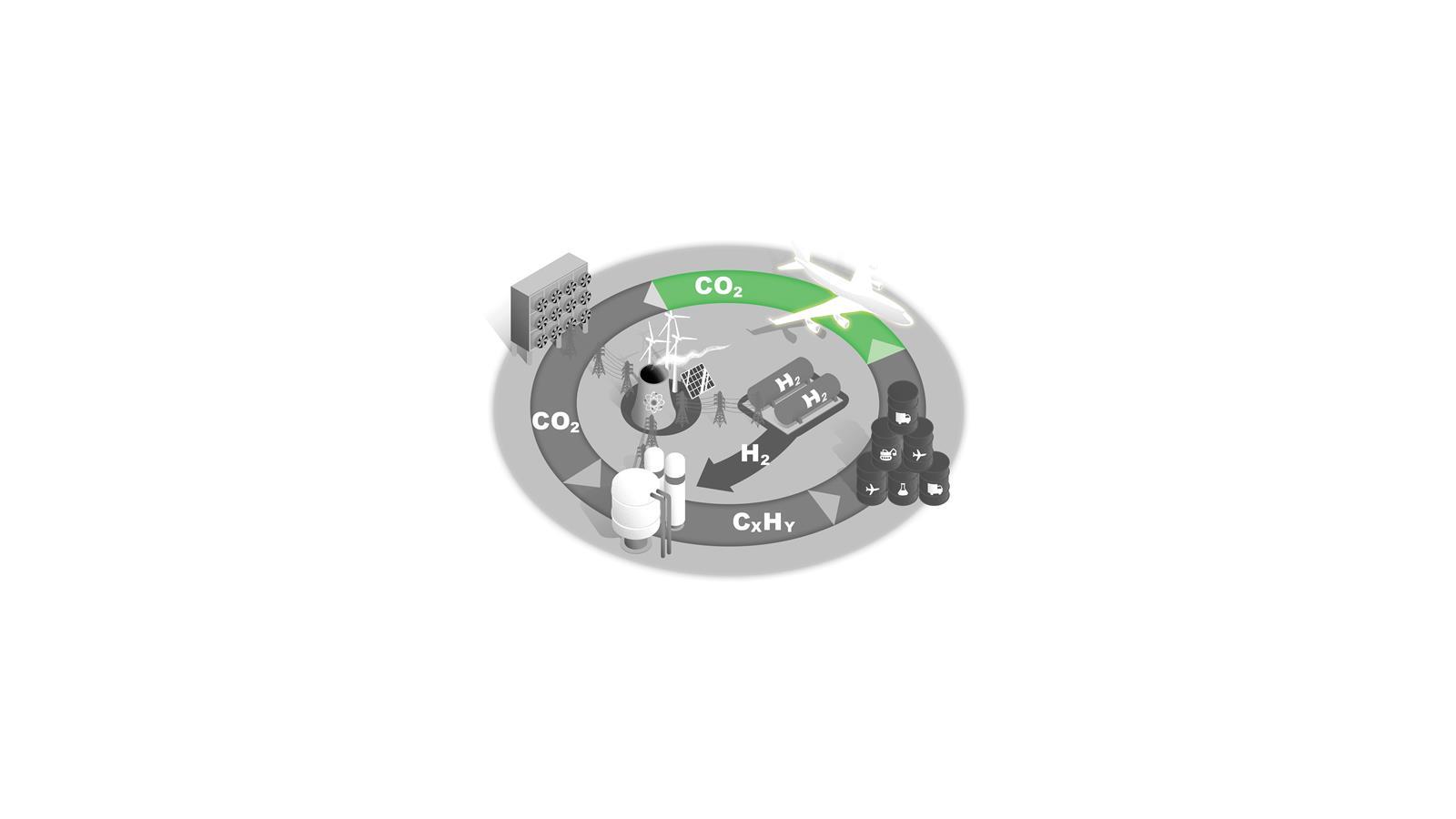
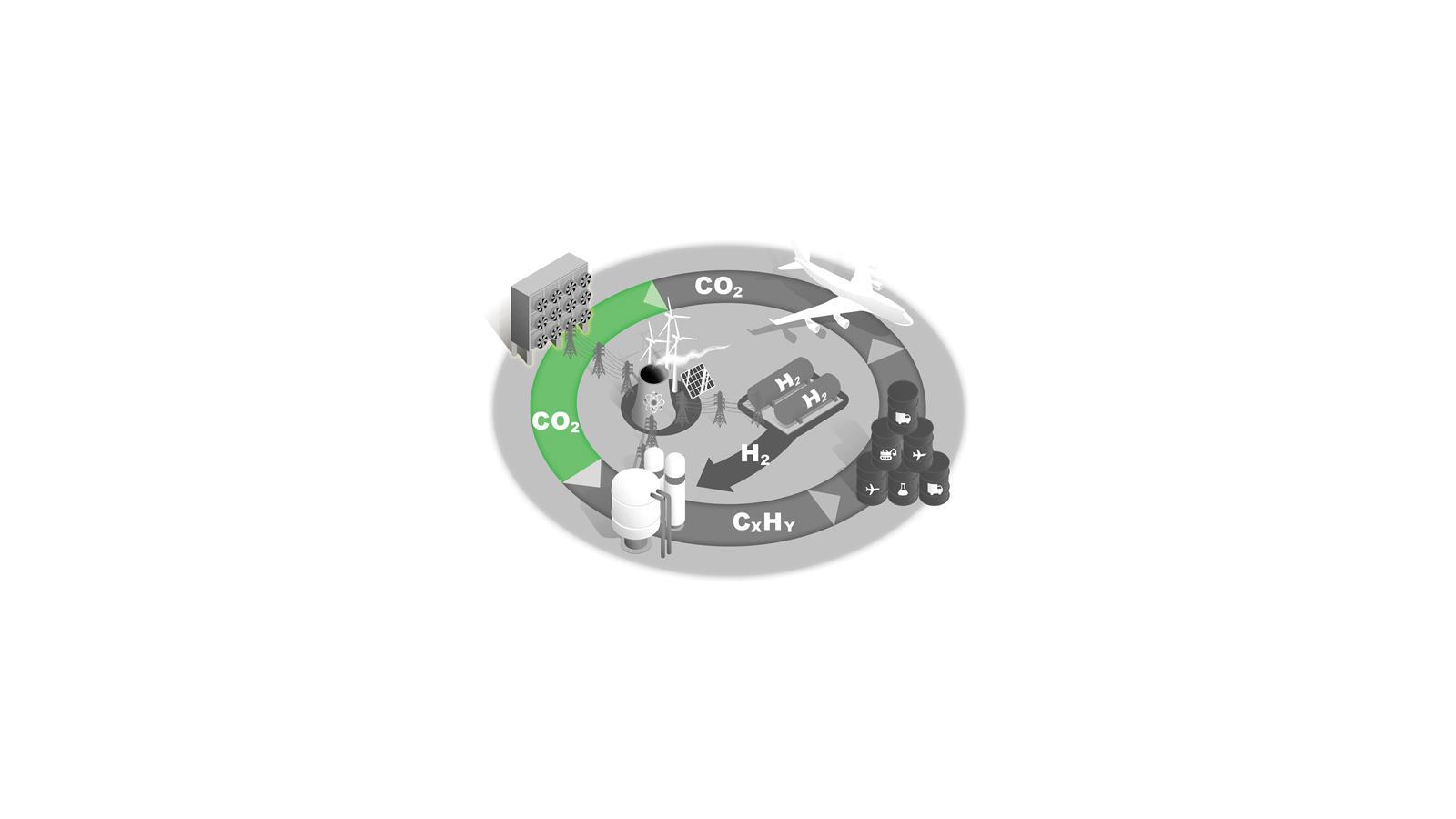
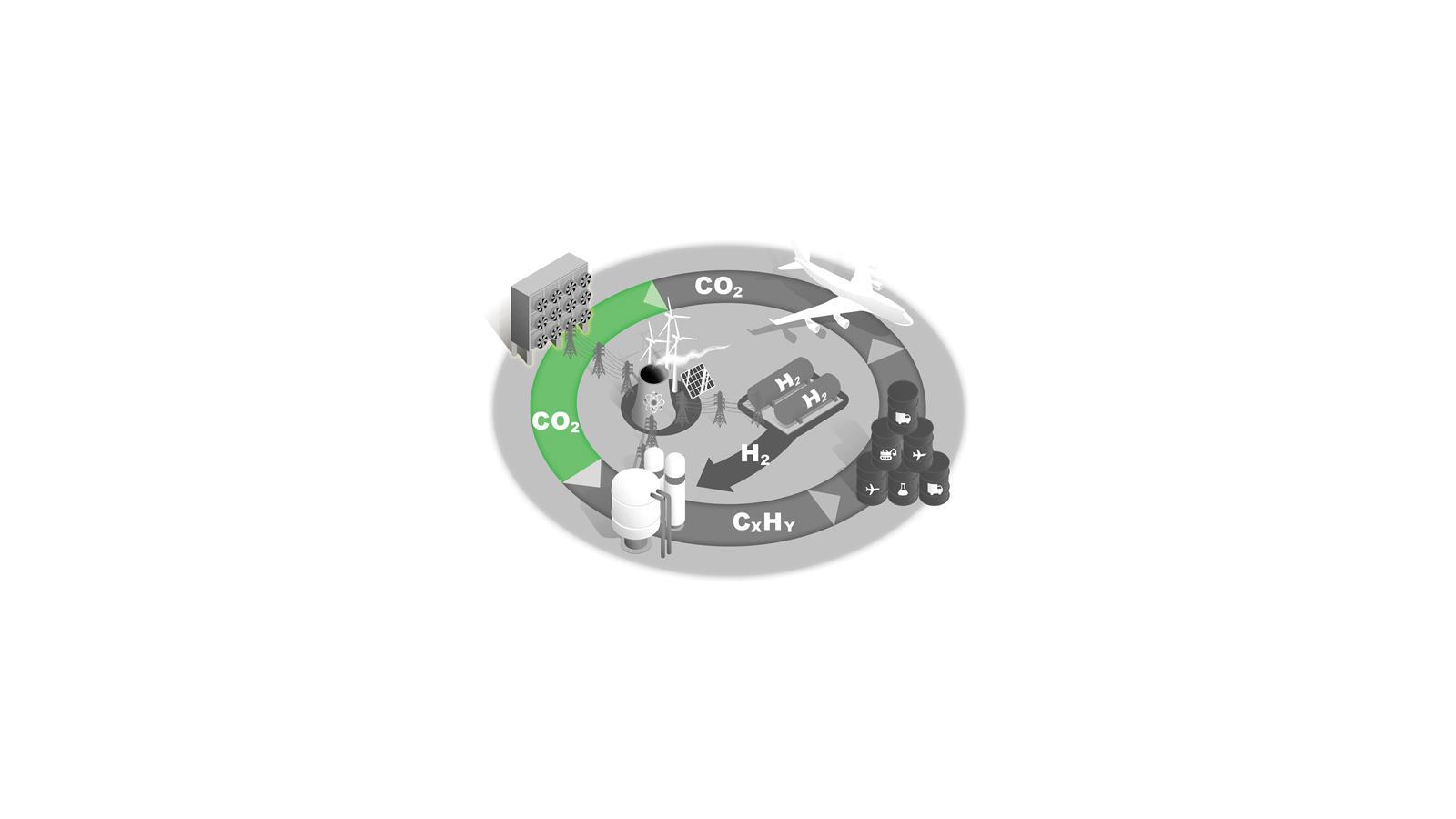
A new carbon cycle
Converting C1 feedstocks such as CO2 and methane into liquid fuels would supply key sectors with sustainable fuels and reduce atmospheric CO2. Each stage presents scientific, technical and economic challenges.
Low-carbon electricity and storage
Decarbonising our energy infrastructure by moving to low carbon sources is central to achieving net zero in every sector.
Blue and green hydrogen
H2 is a key commodity in future net zero economies and will be subject to competing demands as both an energy carrier and a feedstock.
C1 feedstocks to fuels
Fischer–Tropsch synthesis is the only established technology for converting C1 feedstocks to liquid hydrocarbon fuels. Other technologies are in development.
Liquid fuels
Synthetic hydrocarbon fuels can be used in sectors that are the most difficult to electrify, such as heavy machinery and heavy goods vehicles.
The chemicals sector would be another priority user with its established plant and infrastructure based on liquid hydrocarbon feedstocks.
This fuel source could also be key to decarbonising air travel.
Aircraft
The aerospace industry is difficult to decarbonise and this sector therefore needs a sustainable liquid hydrocarbon fuel as a step in its net zero transition.
Direct air capture
Removing CO2 from the atmosphere is essential for net zero. Capturing diffuse CO2 from the air is a formidable thermodynamic challenge, however.
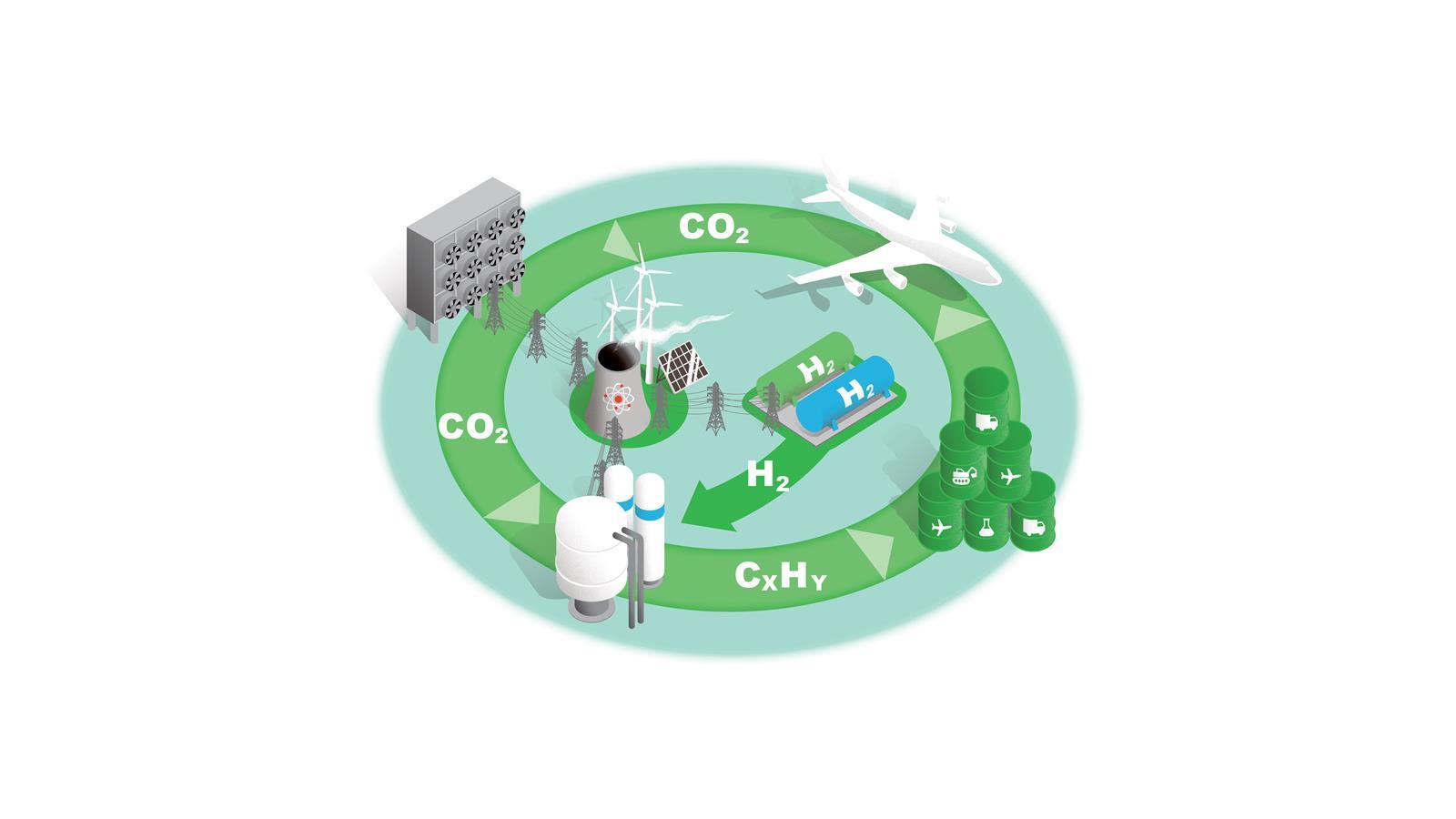
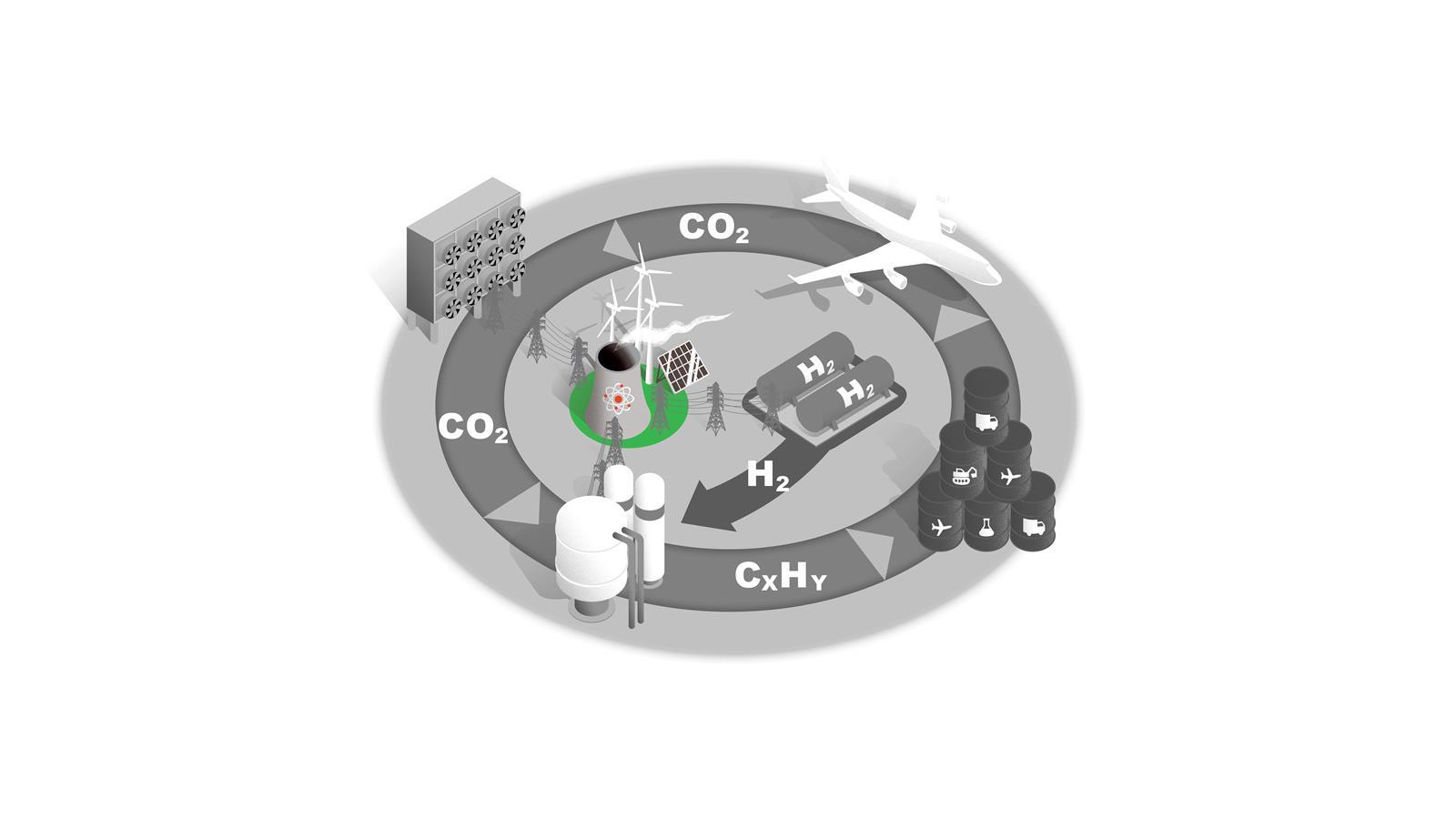
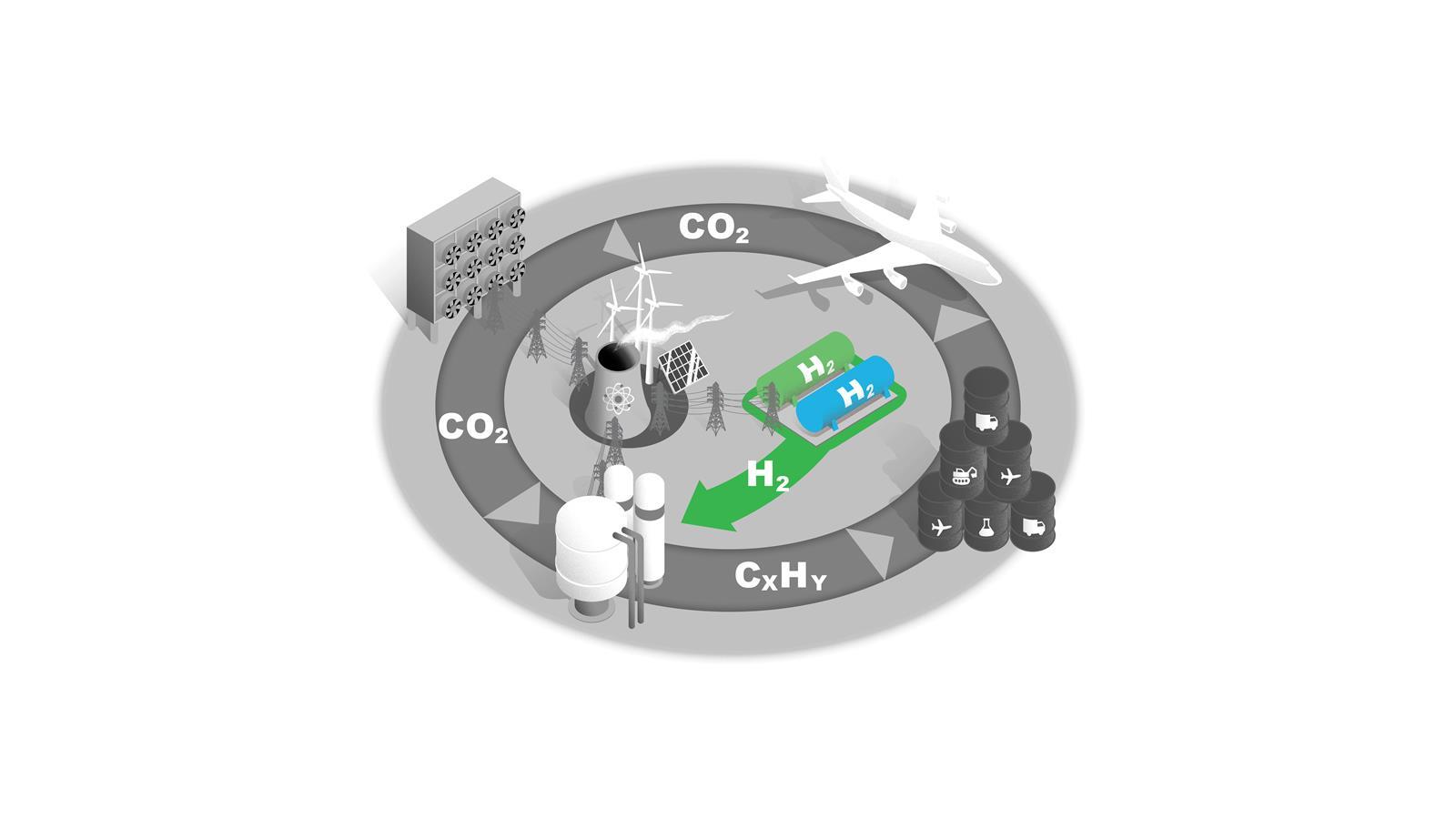
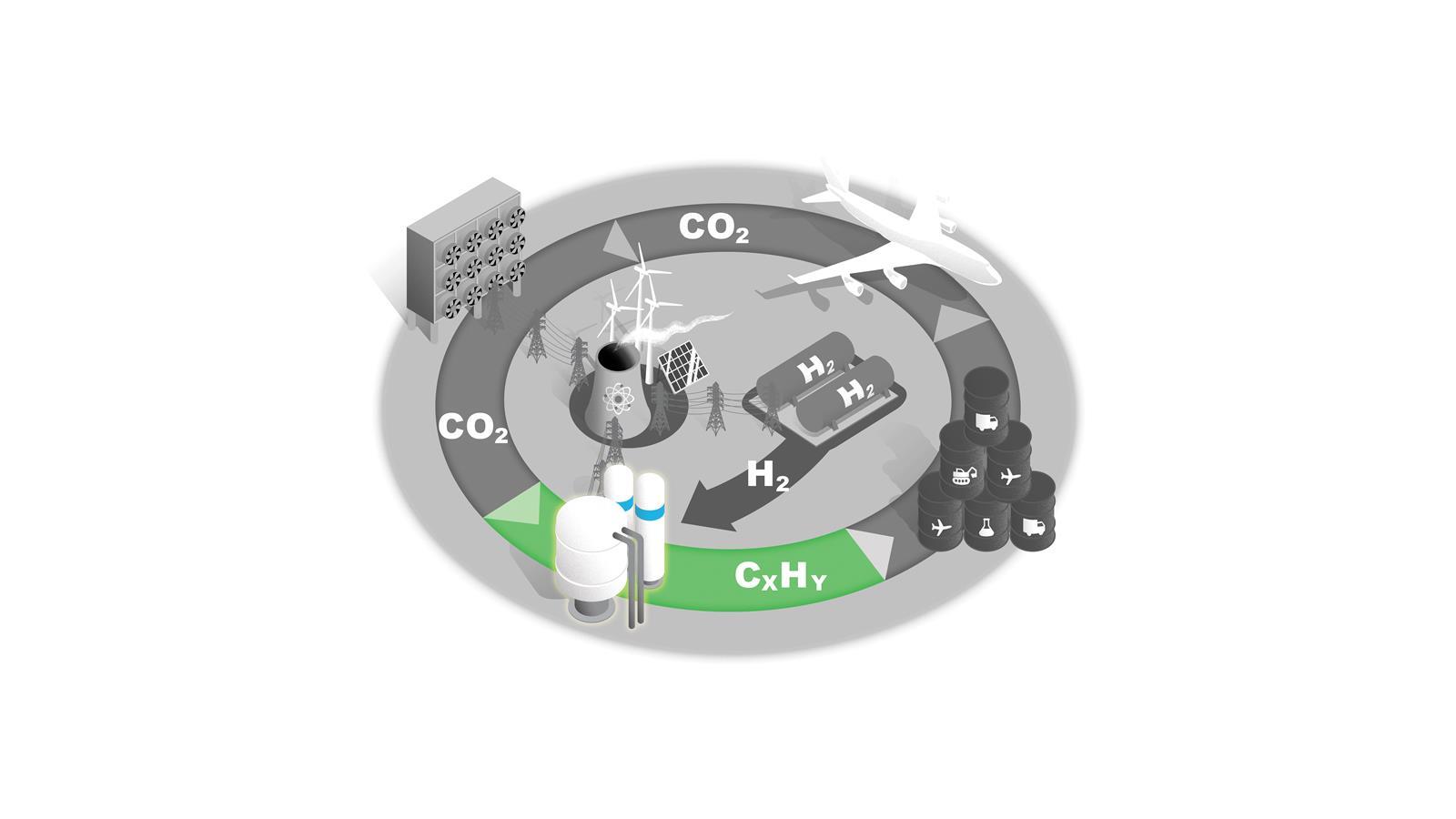
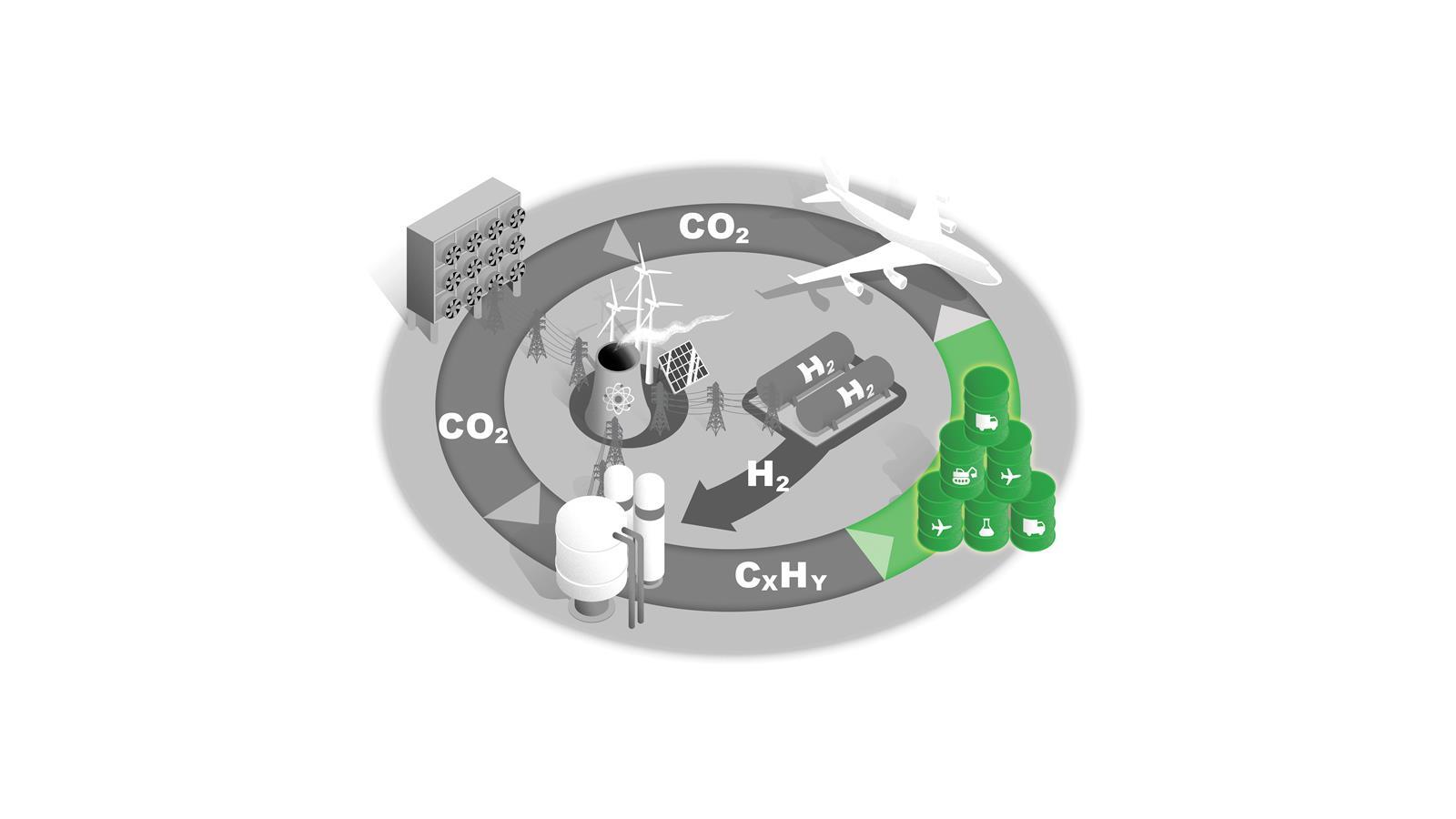
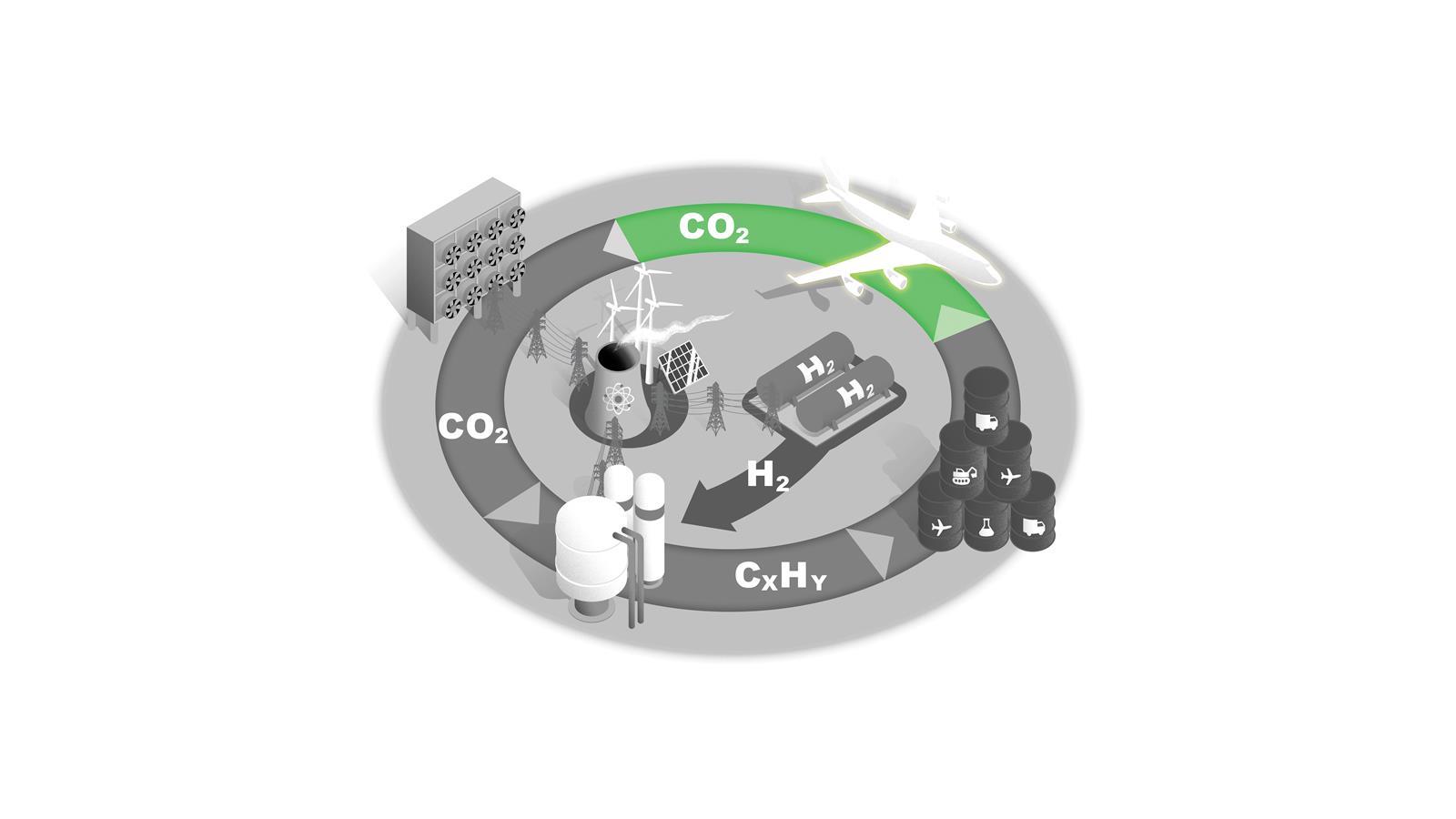
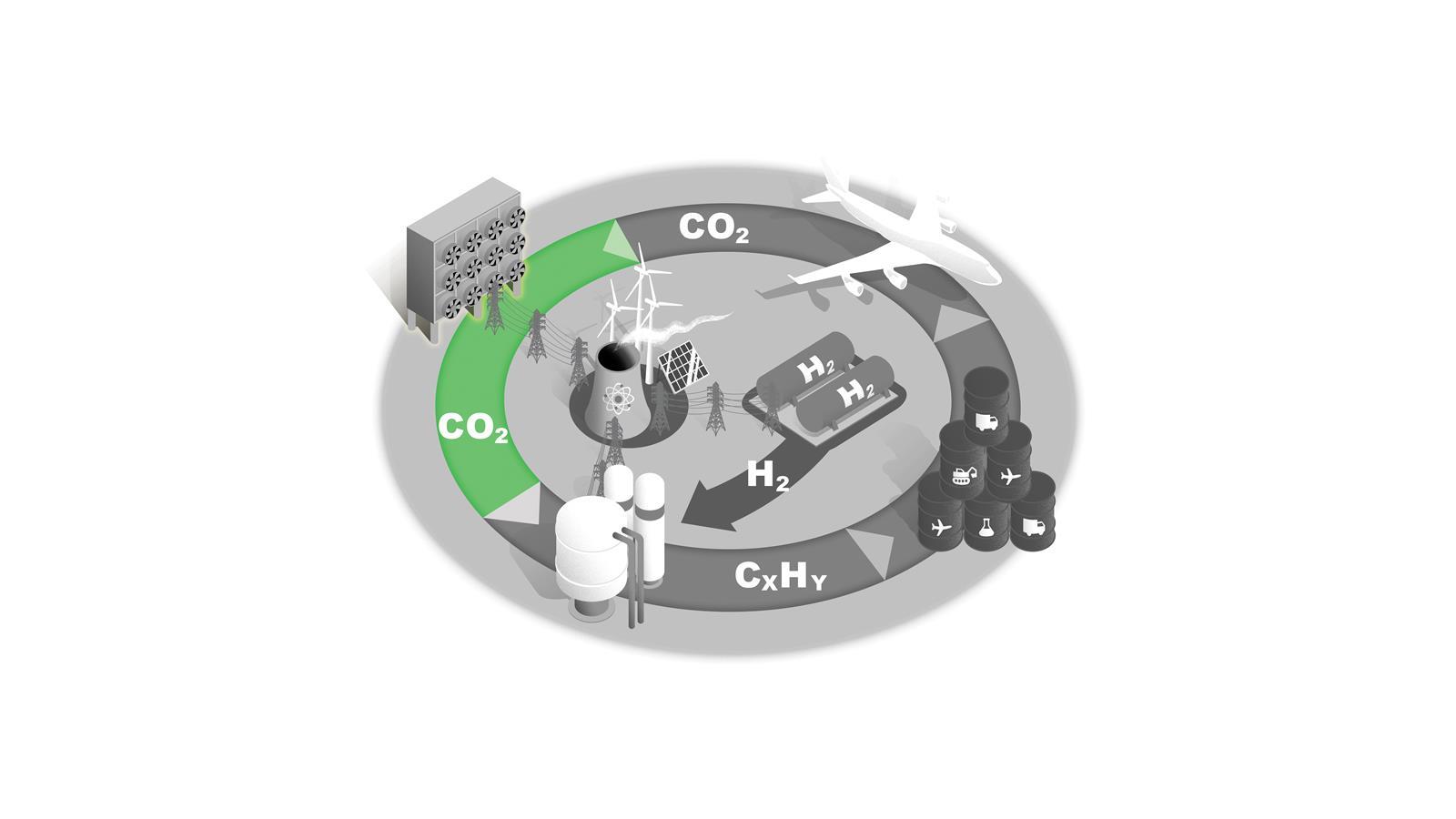
A new carbon cycle
Converting C1 feedstocks such as CO2 and methane into liquid fuels would supply key sectors with sustainable fuels and reduce atmospheric CO2. Each stage presents scientific, technical and economic challenges.
Low-carbon electricity and storage
Decarbonising our energy infrastructure by moving to low carbon sources is central to achieving net zero in every sector.
Around half of the UK’s current electricity production comes from low-carbon sources, and as new technologies and infrastructure that enable net zero are established demand will only increase.
For the C1 to fuels process illustrated here, electrolysis, CO2 capture and chemical processes all require a significant expansion of low-carbon electricity production.
Blue and green hydrogen
H2 is a key commodity in future net zero economies and will be subject to competing demands as both an energy carrier and a feedstock.
In the near term, blue hydrogen made from natural gas with carbon capture is the most scalable option, but this process has low efficiency and is likely to have residual greenhouse gas emissions. Longer term, increasing production capacity of green H2 via electrolysers will be needed.
C1 feedstocks to fuels
Fischer–Tropsch synthesis is the only established technology for converting C1 feedstocks to liquid hydrocarbon fuels. Other technologies are in development.
In all cases, the process is only economically viable with carbon taxes on competing products or by improving its efficiency by developing catalysts that increase specificity and reduce energy consumption.
Liquid fuels
Synthetic hydrocarbon fuels can be used in sectors that are the most difficult to electrify, such as heavy machinery and heavy goods vehicles.
The chemicals sector would be another priority user with its established plant and infrastructure based on liquid hydrocarbon feedstocks.
This fuel source could also be key to decarbonising air travel.
Aircraft
The aerospace industry is difficult to decarbonise and this sector therefore needs a sustainable liquid hydrocarbon fuel as a step in its net zero transition.
There are also significant embedded costs in the stock of current fleets that are expected to last for decades. And it is a highly regulated industry so any changes to aircraft design (for new fuel types, for example) require new regulations to be developed.
Direct air capture
Removing CO2 from the atmosphere is essential for net zero. Capturing diffuse CO2 from the air is a formidable thermodynamic challenge, however.
One estimate states that capturing 1 tonne of CO2 would require 1200kWh of electricity just for this first step. Only pilot plants exist currently and significant development of the technology is needed.
Up in the air
Take aviation fuel as an example. Jet fuel is a highly refined kerosene used by planes with gas-turbine engines. In 2019, the UK aviation sector consumed 12.3 million tonnes of jet fuel, the third largest use in the world. In the long-term, new fleets of hydrogen-fuelled aircraft could make carbon-free air travel a reality. But in the near and medium term, our current fleets will continue to run on liquid-hydrocarbon fuels and these must come from sustainable sources.
The scale of the decarbonisation challenge is huge: in 2013, 513Mt of fossil fuel feedstocks were used in the chemical and petrochemical sectors
There are a number of different options for replacing fossil fuel based jet fuel. A promising one prima facie is biofuels: various chemical pathways and feedstocks can convert biomass to fuel, including Fischer–Tropsch reformation of syngas, alcohol and hydroprocessed oil. Yet producing the biomass they depend on poses a land-use problem. A recent assessment found that, depending on the biomass source, it would require somewhere between 30–68% of UK agricultural land to grow the feedstocks needed to produce those 12.3 million tonnes of jet fuel.2 Another estimate by a University of Cambridge team suggests we would need 120% of the world’s food production to supply the feedstocks needed to scale the production of sustainable aircraft fuel to 2019 fossil-fuel usage levels. This is the food–fuel tension. Using waste streams can ease some of that tension, but those streams are typically very heterogeneous and much more challenging to process.
If biomass is not viable as our carbon feedstock, another option is bioproduction. However, the current bioproduction systems for chemicals are primarily based on sugar-containing substrates. So again these are dependent on biomass and substrate sustainability and affordability becomes a challenge.
This illustrates the nature of the systems challenge. In a decarbonising world, the need for biomass outstrips its supply with competing pressures from energy, materials and other industrial sectors. For the EU, it has been estimated that the there is a 40–70% gap between biomass supply and estimate demand in current climate scenarios.3 It is not possible to import more, cultivate more or further exploit waste streams. Moreover, depending on its use, biomass may not be sustainable nor carbon neutral.
Where do we get carbon from for fuels and materials if not fossil fuels or biomass?
Such systems thinking also applies to the idea of using hydrogen as an aviation fuel. You can make hydrogen from electrolysis of water using renewable energy (green hydrogen), but to produce the equivalent of 12Mt of aviation fuel would require 207–290TWh of electricity2 – last year the UK generated just over 325TWh in 2022 in all. We could use blue hydrogen from the steam reformation of methane (with an efficiency of 60-65%), but this also produces CO2 that has to be captured and stored. There is also water consumption to consider: to generate 1kg of hydrogen from electrolysis requires 10 litres of deionised water; natural gas reforming with CO2 capture requires 25 litres. Arguably, hydrogen has better roles elsewhere in the net zero journey, such as in decarbonising the chemical industry as a clean (depending on how it is made) source of high temperature heat as well as a key reactant.
A closed carbon loop
This leads to a generalisable problem: where do we get carbon from for fuels and materials if not fossil fuels or biomass? The answer is to use the abundant C1 carbon sources that are all around us: the very CO2 and methane responsible for global warming.
This can be achieved by capturing them from the atmosphere or intercepting the exhaust gases of power stations, cement kilns or other industries. Using concentrated CO2 from flues as a feedstock for fuels is clearly more attractive from a kinetic point of view; however, it is unlikely to help reduce emissions. If the carbon in captured CO2 started out as a fossil fuel or in carbonate rocks then converting it into a fuel that is later burned in an aircraft or vehicle leads to the same net result: an increase in the stock of carbon in the atmosphere. We have merely prolonged the journey. If the captured CO2 is instead used to make durable plastics or construction materials that are retained in a circular economy, that would reduce emissions, but we are far from that situation at present.
Affordably fixing carbon from the atmosphere is therefore an essential challenge for the chemical sciences in the 21st century. It would give us the starting point for complex molecules without the need to place more demands on agricultural land, and also the potential to reverse some of the accumulation of CO2 in the atmosphere.
However, it is also very difficult to do: CO2 is diffuse and unreactive in the atmosphere, and if we need to expend enormous amounts of low carbon energy to drive this process (one estimate is 1200kWh per tonne for the capture alone) then it will be expensive and unachievable.4 All of the technologies that could deliver C1 to fuel pathways (see box The chemical challenges) therefore have a common need for innovation in catalysis to improve their efficiency.
The chemical challenges
There are well-established routes from C1 sources to fuels, as well as promising early-stage research such as new bioproduction strategies based on biorefinery and electro-biotechnology. In all cases, innovation in catalysis, separation and upgrading of C1 compounds, such as methanol and formate, are vital.
Fischer–Tropsch synthetic pathways are the most developed routes to liquid fuels that do not conflict with food supply or jeopardise the climate. Long chain, unsaturated and aromatic molecules can be made this way, but the process is energy and capital intensive and typically requires costly separation steps. Sodium and sulfur promoted iron catalysts have been identified that improve selectivity over traditional cobalt or iron catalysts.5 Both cobalt carbide nanoprisms and oxide-zeolite-based processes have been shown to deliver even greater yields of desired C2–C4 light olefins. A pilot plant based on the latter process has shown promise, but the reaction still produces a mix of products including waste CO2.6
Electroreduction of carbon monoxide or carbon dioxide can be much more selective and efficient, with candidate systems using copper particles on a modified carbon support, though improved stability is required for commercialisation.7,8
Hydrogenation of CO2 to methanol and formate can be performed by Cu/ZnO/Al2 O3 catalysts, Cu/ZnO, or noble metals such as indium, gold, platinum and palladium.5 These C1 compounds are valuable chemical feedstocks, can store energy at high density and could be used to generate electricity in fuel cells. Methanol-to-olefins reactions, using a zeolite catalyst, also give a sustainable route to polymers.9
Anaerobic digestion of agricultural wastes already produces biomethane at scale, a feedstock that could be upgraded. Oxidative and non-oxidative coupling of methane reactions hold promise using platinum, molybdenum oxide supported on zeolites, single iron embedded in a silica matrix, or lithium magnesium oxide, but the challenges of catalyst lifespan and the need to avoid over-oxidation to CO2 persist.
The scale of the decarbonisation challenge is huge: in 2013, 513Mt of fossil fuel feedstocks were used in the chemical and petrochemical sectors.10 Chemistry is part of the solution, but we must consider the whole system: the complexities and limitations in establishing these new carbon flows mean that demand side measures to reduce consumption are also needed, alongside improving our material and resource efficiency. Only through combining all these measures will we keep within our carbon budgets.
For the chemistry community, this is a clarion call to embrace the innovation requirements of the net zero transition – to take the useless and make it useful.
References
1 P Gabrielli et al,One Earth, 2023, 6, 682 (DOI: 10.1016/j.oneear.2023.05.006)
2 Net Zero aviation fuels – resource requirement and environmental impacts policy briefing, 2023, Royal Society, London
3 EU Biomass use in a net zero economy – a course correction for EU biomass, Material Economics, 2021
4 A P Zeng,Biotechnol. Adv., 2019, 37, 508 (DOI: 10.1016/j.biotechadv.2019.01.003)
5 Y Liu, D Deng and X Bao, Chem, 2020,6, 2497 (DOI:10.1016/j.chempr.2020.08.026)
6 S Zhao et al, Fuel, 2022, 321, 124124 (DOI:10.1016/j.fuel.2022.124124)
7 R Chen et al, Angew. Chem. Int. Ed., 2020,59, 154 (DOI: 10.1002/anie.201910662)
8 E Andreoli, Nat. Catal., 2021,4, 8 (DOI: 10.1038/s41929-020-00568-9)
9 P Tian et al, ACS Catalysis, 2015,5, 1922 (DOI: 10.1021/acscatal.5b00007)
10 P G Levi and J M Cullen,Environ. Sci. Technol., 2018, 52, 1725 (DOI: 10.1021/acs.est.7b04573)
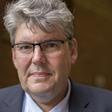
No comments yet