Philip Ball gets in a twist about DNA
How much of biology can be explained by physics? Asking that question is often a good way of starting a fight, but there is at least one issue for which the argument seems settled: the mechanical properties of DNA have an undoubted influence on biological functions. DNA mechanics seems crucial, for example, to the tight packaging of genomic material inside viruses (where it seems poised like a wound spring for injection into infected cells), the folding-up of chromosomes, and the binding to DNA of proteins that regulate the readout of genetic information (transcription).
The storage and transmission of genetic information thus depends not just on the sequence of nucleotide bases, like beads on a string, but also on ‘engineering’ the proper flexibility, springiness and coiling in the twisted necklace.
Mechanically speaking, DNA is no ordinary polymer. Relatively stiff polymers have traditionally been treated as ‘elastic rods’, a little like a length of metal cable. But DNA seems to bend and twist more readily than this picture would predict.1 Stranger still: if you wring out a cloth it gets shorter, yet twisting DNA makes it elongate2 - a result of the tilting that this induces in the base pairs zipping the double helix together.
The extra-bendiness of DNA is perhaps a biological necessity, because it has to squeeze into some tight spots. In some bacterial viruses (bacteriophages) the genetic material has to be crammed into a protein shell just 30 nm or so across, demanding that it be bent far more tightly than thermal fluctuations alone would enable. Bacteriophages possess powerful protein motors for spooling DNA into crammed spaces, which generate forces a factor of 100 or so greater than those typically exerted by the muscle motor protein myosin.
One of the most important aspects of DNA mechanics is its role in transcription and replication. In eukaryotes (cells in which the genome is packaged inside a nucleus), DNA is wound around disc-shaped proteins called histones, typically just 7nm or so in diameter. The protein machinery of transcription can only access genes by loosening these coils. Winding DNA onto histones costs bending energy, which is compensated by the interaction between the negatively charged double helix and positively charged groups on the histone proteins.
It seems that this energetic balance is delicately poised: it is just favourable enough to ensure that the DNA gets spontaneously wound, but not so much that it can’t be unwrapped fairly easily.3
The helical structure of double-stranded DNA creates a potential problem for proteins that step along the chain during transcription. Either they must corkscrew around the coil as they go, or they must push the coiling ahead of them, making the upstream helix overwound (positively supercoiled) and the downstream part underwound (negatively supercoiled). In practice, the latter generally happens. But this supercoiling also has an energy cost, and affects how other proteins bind to regulate transcription and replication. So it needs to be carefully managed in the cell. In general this is done by enzymes called topoisomerases, which can nick the double helix to relax overwinding.
It’s important to know, then, how readily supercoiling happens and gets propagated along the chain. Nynke Dekker of the Delft University of Technology in the Netherlands and her coworkers have recently studied this issue for single molecules on DNA attached at one end to a solid surface and at the other to a magnetic bead.4 The researchers introduced supercoiling by magnetically rotating the bead, and followed how the strands twist by applying a sudden stretching force: they used optical tweezers to pull the bead away from a magnet, and then switched the light off so the bead sprang back.
Supercoiling creates twisted loops called plectonemes that stick out from the strand. These are familiar from twisted telephone cables (remember them?), where they can be removed by stretching the wire.
Dekker and colleagues asked whether the rate at which supercoiled DNA gets longer when pulled is dominated by the unwinding of plectonemes or by elastic stretching of the strand itself. It seems plectonemes can relax and unwind very quickly, so the elongation depends mostly on how hard it is to stretch a ‘pure’ double helix.
Dekker and colleagues have also used single-molecule manipulation to figure out how the anticancer drug topotecan kills tumour cells by interfering with topoisomerase enzymes.5 Topotecan makes topoisomerase stick covalently to DNA, clamping the double helix and preventing strand separation for replication. But there is a second lethal effect too: the drug hinders the enzyme’s ability to remove positive supercoils, so that these pile up in front of the replication machinery, causing it to stall and fatal DNA lesions to accumulate.
References
1 T E Cloutier & J Widom, Proc. Natl Acad. Sci., 2005, 102, 3645 (DOI:10.1073/pnas.0409059102)
2 T Lionnet et al, Phys. Rev. Lett., 2006, 96, 178102 (DOI: 10.1103/PhysRevLett.96.178102)
3 H G Garcia et al, Biopolymers, 2006, 85, 115 (DOI:10.1002/bip.20627)
4 A Crut et al, Proc Natl Acad. Sci., DOI:10.1073/pnas.0700333104
5 D A Koster et al, Nature, 2007, 448, 213 (DOI:10.1038/nature05938)
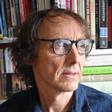
No comments yet