We need to stop viewing proteins as static and embrace their dynamism
Time, the physicist John Wheeler famously said, is nature’s way of preventing everything from happening at once. Yet our instincts are to squish events back into timeless superposition. From the simultaneous comic-strip of the Bayeux Tapestry to the summed statistics of football matches, we tend to convert complex, dynamic processes into static images and averages. Technologies that capture temporal change are relatively recent, and Louis Daguerre’s earliest photographs of street scenes, in which ephemeral pedestrians vanish during the long exposure, offer a metaphor for how time-averaging can wash away crucial details.
I’m just trying to make chemists feel better here – for the molecular sciences have long struggled with the dynamical viewpoint. The first glimpse into the molecular world, provided by x-ray crystallography in the early 20th century, made chemistry a realm of Platonic structure, encouraging a static mindset that persists today. Everyone knows that drawing static molecular structures is a matter of necessary convenience, but habit eventually infects thinking. For biomolecules in particular, the crystallographic worldview fostered by the likes of Pauling, Bernal, Hodgkin, Franklin and Kendrew made the lock-and-key concept of enzyme action a compelling image.
Freeze frame
Of course, there’s a great deal that’s correct in this picture. Most understanding of protein function has come from studies of their structure. We can see how ligands fit into clefts, how membrane proteins make pores for small molecules to pass, how large assemblies like the ribosome and photosystems consist of elegantly interlocking parts. But life isn’t a crystal frozen in time. It is a process, unfolding such that no picosecond exactly resembles any other. Just as development is as much about timing as it is about regulatory genes, so molecular cell biology is a world of orchestrated motion.
As with our own pasts, we seem so often constrained to reduce this dynamic story to a series of snapshots. That was how, as ‘femtochemistry’ was pioneered in the 1990s by Ahmed Zewail and others, the time course of simple chemical reactions was generally presented: static instants caught by the rapid strobe of femtosecond laser pulses. We struggle to find good dynamical metaphors, although for proteins the emerging picture is of a molecule wandering over a free-energy landscape. Rather than condense the resulting diversity into a single average, that structural variety is enshrined in an ensemble view. And increasingly it’s recognised that this is sometimes the best way to understand function.
Breaking the lock
Basic biochemistry textbooks have long modified the lock-and-key model, for example with the induced-fit idea that recognises a protein’s binding site changes shape to accommodate a ligand. But that can sound just like a shift from one static form to another. It now seems that dynamics alter the energy landscape on which a reaction takes place. The critical role of dynamics was already becoming recognised in the 1970s and 80s; in 1983 Martin Karplus and Andrew McCammon stated that ‘the static view of protein structure is being replaced by a dynamic picture.’1
There are countless examples. The sensitivity of the protein-digesting enzyme proteinase K to calcium ions, for instance, seems to be caused not by a conformational switch on ion binding but by a change in the dynamical flexibility of the binding site.2 Such a change fits the notion of ‘conformational selection’,3 in which fluctuations allow the protein to explore the entire free-energy landscape. Likewise, the proteins making up the major histocompatibility complex (MHC) that plays a key role in the immune response have their motions ‘tuned’ by the peptides that the complex binds, in ways that might be important for understanding immunity and developing vaccines.4 And very recent simulations by Yang et al5 suggest that the free-energy barrier of binding of amino acid-charged transfer RNA to the ribosome during protein synthesis is influenced by the degree of mobility of a ribosomal peptide appendage denoted L11. The L11 stalk has a flexible region that permits large-scale fluctuations – so mutation might provide a means of tuning the accommodation of the loaded tRNA.
Shifting structures
Allostery – the process in which a binding event at one part of a protein somehow regulates function at a remote region – has long been discussed as if it happens by a series of mechanical shifts akin to a crankshaft. But it might be more profitably seen as a change in the dynamic ensemble of protein conformations.6 That picture is perhaps most forcefully implied by intrinsically disordered proteins,7 which challenge the whole notion of function being tied to structure.
So ‘protein structure’ is perhaps better regarded as a set of conformations, akin to the choreographed poses through which a dancer continually moves. This dynamic description has to embrace the environment too: it’s long been clear that biological macromolecules engage in a delicate dance with their solvent.8 Water ‘injects’ fluctuations into a protein, which in turn dynamically shapes the nearby solvent in ways that can have functional consequences.9
Can all this time-dependent complexity be accommodated in an intuitive picture of how proteins work? That challenge is increasingly urgent, because our view of protein function will become complicated, as well as deepened, by the advent of single-molecule diffraction using x-ray free-electron lasers.10 These techniques – as well as the Nobel-winning cryo-electron microscopy – will supplement conventional crystallography by potentially supplying a view of the entire conformational landscape. I can’t help wondering whether structural biologists might then find themselves searching for a different name.
Time, the physicist John Wheeler famously said, is nature’s way of preventing everything from happening at once. Yet our instincts are to squish events back into timeless superposition. From the simultaneous comic-strip of the Bayeux Tapestry to the summed statistics of football matches, we tend to convert complex, dynamic processes into static images and averages. Technologies that capture temporal change are relatively recent, and Louis Daguerre’s earliest photographs of street scenes, in which ephemeral pedestrians vanish during the long exposure, offer a metaphor for how time-averaging can wash away crucial details.
I’m just trying to make chemists feel better here – for the molecular sciences have long struggled with the dynamical viewpoint. The first glimpse into the molecular world, provided by x-ray crystallography in the early 20th century, made chemistry a realm of Platonic structure, encouraging a static mindset that persists today. Everyone knows that drawing static molecular structures is a matter of necessary convenience, but habit eventually infects thinking. For biomolecules in particular, the crystallographic worldview fostered by the likes of Pauling, Bernal, Hodgkin, Franklin and Kendrew made the lock-and-key concept of enzyme action a compelling image.
Freeze frame
Of course, there’s a great deal that’s correct in this picture. Most understanding of protein function has come from studies of their structure. We can see how ligands fit into clefts, how membrane proteins make pores for small molecules to pass, how large assemblies like the ribosome and photosystems consist of elegantly interlocking parts. But life isn’t a crystal frozen in time (unless we’re talking about this year’s Nobel prize). It is a process, unfolding such that no picosecond exactly resembles any other. Just as development is as much about timing as it is about regulatory genes, so molecular cell biology is a world of orchestrated motion.
As with our own pasts, we seem so often constrained to reduce this dynamic story to a series of snapshots. That was how, as ‘femtochemistry’ was pioneered in the 1990s by Ahmed Zewail and others, the time course of simple chemical reactions was generally presented: static instants caught by the rapid strobe of femtosecond laser pulses. We struggle to find good dynamical metaphors, although for proteins the emerging picture is of a molecule wandering over a free-energy landscape. Rather than condense the resulting diversity into a single average, that structural variety is enshrined in an ensemble view. And increasingly it’s recognised that this is sometimes the best way to understand function.
Breaking the lock
Basic biochemistry textbooks have long modified the lock-and-key model, for example with the induced-fit idea that recognises a protein’s binding site changes shape to accommodate a ligand. But that can sound just like a shift from one static form to another. It now seems that dynamics alter the energy landscape on which a reaction takes place. As early as 1983, Martin Karplus and Andrew McCammon stated that ‘the static view of protein structure is being replaced by a dynamic picture.’
There are countless examples. The sensitivity of the protein-digesting enzyme proteinase K to calcium ions, for instance, seems to be caused not by a conformational switch on ion binding but by a change in the dynamical flexibility of the binding site. Such a change fits the notion of ‘conformational selection’, in which fluctuations allow the protein to explore the entire free-energy landscape. Likewise, the proteins making up the major histocompatibility complex (MHC) that plays a key role in the immune response have their motions ‘tuned’ by the peptides that the complex binds, in ways that might be important for understanding immunity and developing vaccines. And very recent simulations by Yang et al suggest that the free-energy barrier of binding of amino acid-charged transfer RNA to the ribosome during protein synthesis is influenced by the degree of mobility of a ribosomal peptide appendage denoted L11. The L11 stalk has a flexible region that permits large-scale fluctuations – so mutation might provide a means of tuning the accommodation of the loaded tRNA.
Shifting structures
Allostery – the process in which a binding event at one part of a protein somehow regulates function at a remote region – has long been discussed as if it happens by a series of mechanical shifts akin to a crankshaft. But it might be more profitably seen as a change in the dynamic ensemble of protein conformations. That picture is perhaps most forcefully implied by intrinsically disordered proteins, which challenge the whole notion of function being tied to structure.
So ‘protein structure’ is perhaps better regarded as a set of conformations, akin to the choreographed poses through which a dancer continually moves. This dynamic description has to embrace the environment too: it’s long been clear that biological macromolecules engage in a delicate dance with their solvent. Water ‘injects’ fluctuations into a protein, which in turn dynamically shapes the nearby solvent in ways that can have functional consequences.
Can all this time-dependent complexity be accommodated in an intuitive picture of how proteins work? That challenge is increasingly urgent, because our view of protein function will become complicated, as well as deepened, by the advent of single-molecule diffraction using x-ray free-electron lasers. These techniques – as well as the Nobel-winning cryo-electron microscopy – will supplement conventional crystallography by potentially supplying a view of the entire conformational landscape. I can’t help wondering whether structural biologists might then find themselves searching for a different name.
References
1 M Karplus & J A McCammon, Annu. Rev. Biochem. 1983, 53, 263 (DOI: 10.1146/annurev.bi.52.070183.001403)
2 L-Q. Yang et al., J. Biomol. Struct. Dynam. 2014, 32, 372 (DOI: 10.1080/07391102.2013.770372)
3 J Foote & C Milstein, Proc. Natl Acad. Sci. USA, 1994, 91, 10370
4 C M Ayres, S A Corcelli & B M Baker, Front. Immunol., 2017, 8, 935 (DOI: 10.3389/fimmu.2017.00935)
5 H Yang, J K Noel & P C Whitford, J. Phys. Chem. B, 2017, 121, 10593 (DOI: 10.1021/acs.jpcb.7b06828)
6 H N Motlagh, J O Wrabl, J Li & V J Hilser, Nature, 2014, 508, 331 (DOI: 10.1038/nature13001)
7 P E Wright & H J Dyson, Nat. Rev. Mol. Cell Biol., 2015, 16, 18 (DOI: 10.1038/nrm3920)
8 P W Fenimore et al., Proc. Natl. Acad. Sci. USA, 2002, 99, 16047 (DOI: 10.1073/pnas.212637899)
9 M Grossman et al., Nat. Struct. Mol. Biol., 2011, 18, 1102 (DOI: 10.1038/nsmb.2120)
10 J C Spence, Int. Un. Crystallogr. J., 2017, 4, 322 (DOI: 10.1107/S2052252517005760)
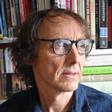
No comments yet